Why DUNE? Searching for the origin of matter
Part I in our series exploring the science goals of the international Deep Underground Neutrino Experiment
Why does matter exist? It may seem like a strange question, but according to current models of the early universe, matter shouldn’t exist.
“According to what we know about the laws of physics, the amount of matter in the universe should be, effectively, zero,” said André de Gouvêa, a theoretical physicist with the DUNE collaboration and professor at Northwestern University.
In physics, the discrepancy between what we see—a universe filled with galaxies and a planet teeming with life—and what models predict we should see—absolutely nothing—is called the “matter-antimatter asymmetry problem.” The international Deep Underground Neutrino Experiment, or DUNE, hosted by the Department of Energy’s Fermilab and to be built at Fermilab and Sanford Lab, seeks to solve this problem, which has dogged physicists for nearly a century.
A universe-sized problem
Despite what the models predict, we find ourselves amidst a universe replete with matter. Everything we see around us is made from just a few types of fundamental particles. Combined, they form protons and neutrons which join up with electrons to form atoms, which in turn bind to make molecules, building ever larger.
But these key ingredients are only half the story.
In the 1930s, physicists discovered “antiparticles” that mirror the fundamental particles. Identical in nearly every way, except with reversed charge, these equal yet opposite particles are called antimatter. Just like matter particles, antimatter particles could combine to build bigger and bigger units of antimatter—if they ever survived long enough do to so.
Although matter and antimatter particles are nearly indistinguishable, the two forms do not coexist peacefully. When antimatter comes into contact with regular matter, particles and antiparticles immediately annihilate, leaving pure energy in their wake.
This complete, mutual annihilation is the impetus of the matter-antimatter asymmetry problem. Our current models dictate that the Big Bang created equal parts matter and antimatter. Within a second, all the matter and antimatter should have met and annihilated, leaving behind a universe with nothing but energy in the form of light.
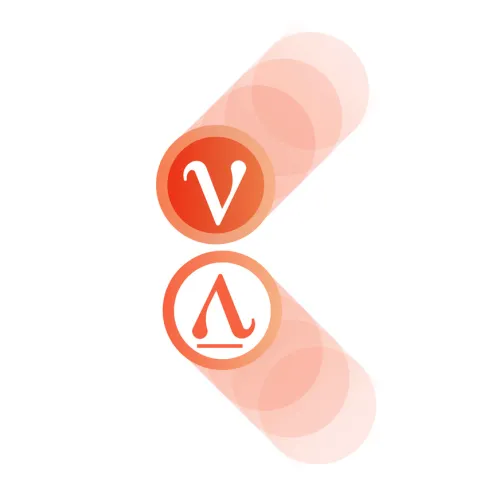
“The problem is, if we take our favorite model and calculate the evolution of the universe, we get a prediction that is completely off,” de Gouvêa said. “There should not be any matter in the universe we live in today.”
We know, of course, that this didn’t happen. We live in a matter-dominated universe with swirling galaxies, innumerable stars and at least one life-sustaining planet. Somehow, about one billionth of the total amount of matter created in the Big Bang managed to evade annihilation and fill the universe with the matter we see today. Thus, the matter-antimatter asymmetry problem.
Physicists believe there is an undiscovered mechanism, hidden in the wrinkles of nature’s laws, that gave matter an initial advantage over antimatter. And for nearly a century, they’ve been trying to pinpoint it.
A crack in nature’s symmetry
Because matter and antimatter are mirror images of each other, physicists assumed that the laws of nature applied to both matter particles and antimatter particles in the exact same way. In physics, this type of equality is called a “symmetry.”
According to this idea, weak and strong forces should bind particles and antiparticles without discrimination. Gravity should pull on antimatter with the same force it exerts on matter. Magnets should attract oppositely charged particles and antiparticles with the same gusto. In fact, an entire universe made of antimatter should look identical to the one we live in today.
This assumption of a perfect symmetry among the fundamental building blocks of the universe held true until the 1960s, when James Cronin and Val Fitch made the shocking discovery that, in a very specific case, the universe treats matter slightly different than antimatter.
Their Nobel Prize-winning experiment examined the way that quarks (fundamental particles that make up protons and neutrons) and antiquarks (their corresponding antiparticles) interacted with the weak force. Rather than treating quarks and antiquarks the same way, the weak force favored quarks in an infamous violation of what is called the Charge Parity (CP) symmetry.
In other words, the universe had revealed a slight preference for matter over antimatter.
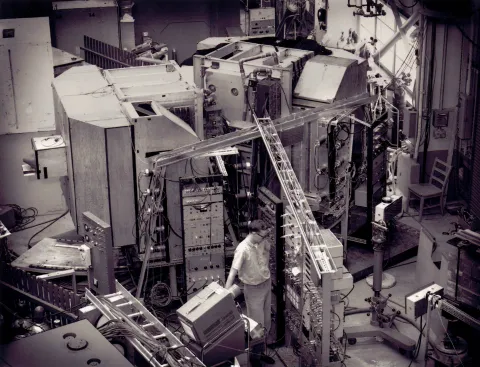
This discovery stunned the particle physics community. In the decades that followed, researchers continued to make precision measurements of these decays, combing their data for new physics that might be lurking within this phenomenon. Thirty years after Cronin and Fitch’s discovery, Elizabeth Worcester was making such measurements at Fermilab’s Tevatron with the KTeV experiment.
“In the 1990s, we were studying the same decays in which CP violation was first observed,” said Worcester, who is now a DUNE physics co-coordinator and physicist at Brookhaven National Laboratory.
This glitch in the laws of nature specifically caught the attention of physicists studying the imbalance of matter and antimatter in the universe. Was this violation of CP symmetry the mechanism that allowed some matter to escape annihilation after the Big Bang?
Subsequent experiments combined with more and more sophisticated calculations demonstrated that nature’s unequal treatment of quarks and antiquarks is not quite big enough to account for the gaping discrepancy we see today.
However, scientists think the existence of CP violation is a major clue.
“This violation could mean there is something very fundamental about the laws of nature that we are missing,” de Gouvêa said.
As soon as Cronin and Fitch made their discovery, physicists began to wonder if other fundamental particles broke the same symmetry. Perhaps multiple sources of CP violation, when combined, could explain how so much matter escaped annihilation in the early universe.
By finding another, even bigger crack in this symmetry, physicists aim to prove that the universe has an overarching preference for matter, making our current universe possible.
A ghost-like candidate
If quarks didn’t provide enough CP violation in the early universe, could another category of elementary particles known as neutrinos have provided another way to favor matter over antimatter?
“If you look at everything that we've learned about neutrinos so far, it indicates that CP could be violated in the neutrino sector,” de Gouvêa said. “There is no specific reason to expect it not to be violated.”
Neutrinos are extremely challenging to work with. Trillions of these particles pass through you each second. Their miniscule mass and neutral charge make them almost impossible to detect. Building an experiment to test whether these ghost-like particles violate the CP symmetry is even more ambitious.
“The reason we don't know if neutrinos violate CP symmetry is purely an experimental issue,” said Ryan Patterson, DUNE physics co-coordinator and professor of physics at the California Institute of Technology (Caltech). “Neutrinos could violate CP a lot, but we don’t know yet because the experiments up to this point haven't been sensitive enough.”
One peculiar property of neutrinos, however, makes the DUNE experiment possible. As neutrinos speed through the universe just under the speed of light, they alternate between three different types, or flavors. This process is called oscillation.
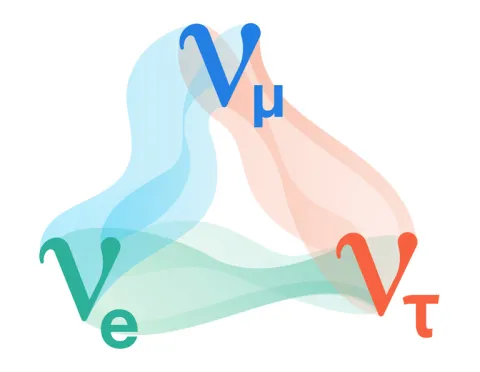
“In regard to neutrinos, we only have one realistic way of measuring CP violation: it will show itself in the way neutrinos oscillate between flavors,” de Gouvêa said.
In principle, the measurement is quite simple, according to de Gouvêa.
“You simply compare a matter process with an antimatter process, and then you ask if they agree,” de Gouvêa said. To measure the CP violation, researchers must compare the oscillations of neutrinos with the oscillations of antineutrinos. If there is a discrepancy in the way they oscillate over a distance, then neutrinos break the symmetry.
The difficult part of the experiment is that neutrino oscillations occur over hundreds of miles. To measure a deviation or discrepancy, researchers would need… well, they would need to build a long-baseline neutrino facility.
Are neutrinos the reason we exist?
The particulars of this universe-sized mystery have guided the design of the aptly named Long-Baseline Neutrino Facility (LBNF), which will house the Deep Underground Neutrino Experiment. Stretching across the Midwest, with infrastructure located at Fermilab in Batavia, Illinois and at Sanford Lab in Lead, South Dakota, the facility allows researchers to measure just how neutrinos and antineutrinos oscillate over long distances.
It works like this: a particle accelerator will generate intense beams of neutrinos and antineutrinos at Fermilab. The beams will travel 800 miles straight through rock and earth – no tunnel needed – to enormous particle detectors located deep underground at Sanford Underground Research Facility (Sanford Lab), where 4,850 feet of rock overburden shield the detectors from unwanted background signals.
During their trip through the Earth’s crust—which takes just four milliseconds—the neutrinos and antineutrinos will oscillate, changing from one flavor into another. Conveniently, the distance between Fermilab and Sanford Lab is ideal for this measurement; by the time the particles arrive at Sanford Lab, their oscillations will be at their peak.
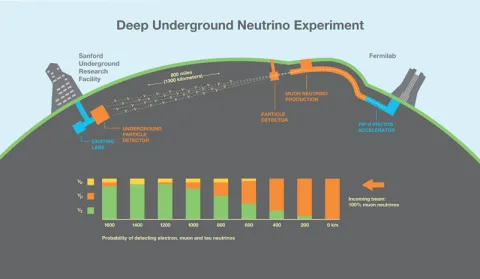
“To get the best measurement, we put the detectors right where we expect the oscillation to be maximal,” Patterson said.
When the beam reaches Sanford Lab, some of the neutrinos and antineutrinos will collide with argon atoms inside the detectors. These collisions result in unique signals. By measuring and comparing hundreds of these signals, researchers will be able to tell if neutrinos and antineutrinos oscillate in different ways – the sure-tell sign of CP symmetry violation – and if so, by how much.
“I think what the neutrinos are going to tell us could change our understanding of nature in a very interesting way,” de Gouvêa said.
So, why DUNE? In a nutshell, it could help scientists answer one of the big unsolved questions in science and give all of us an answer to the reason we—and everything else in the universe—exists.
That, however, is only part of the story. Stay tuned for Part II of our series of stories about the science of DUNE.
Sanford Lab is operated by the South Dakota Science and Technology Authority (SDSTA) with funding from the Department of Energy. Our mission is to advance compelling underground, multidisciplinary research in a safe work environment and to inspire and educate through science, technology, and engineering. Visit Sanford Lab at www.SanfordLab.org.
The Office of Science of the U.S. Department of Energy is the single largest supporter of basic research in the physical sciences in the United States and is working to address some of the most pressing challenges of our time. For more information, please visit energy.gov/science.